Industry perspective on power electronics for electric vehicles
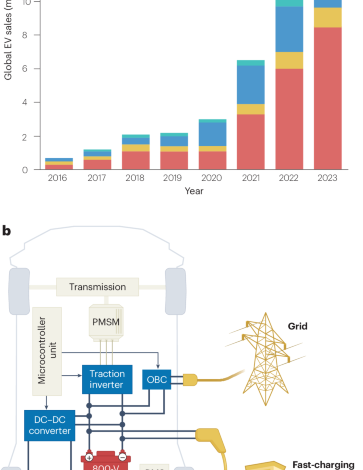
The Oregon Group. Electronic Car Sales Break New Records with Momentum Expected to Continue Through 2023 (International Energy Agency, 2023).
Irle, R. Global EV Sales for 2023. EV Volumes https://ev-volumes.com/news/ev/global-ev-sales-for-2023/ (2024).
Yang, Y. Faster and Stronger: How Will Power Electronics for EVs Reach $9.8 Billion by 2028? Yole Group https://www.yolegroup.com/press-release/faster-and-stronger-how-will-power-electronics-for-evs-reach-9-8-billion-by-2028/ (2023).
McKerracher, C. Battery Bloat Could Backfire on Electric Vehicle Manufacturers. Bloombergnef https://about.bnef.com/blog/battery-bloat-could-backfire-on-electric-vehicle-manufacturers/ (2023).
Baliga, B. J. Enhancement- and depletion-mode vertical-channel m.o.s. gated thyristors. Electron. Lett. 15, 645–647 (1979).
Takeda, T. et al. 1200 V trench gate NPT-IGBT (IEGT) with excellent low on-state voltage. In IEEE International Symposium on Power Semiconductor Devices and ICs (ISPSD) 75–79 (IEEE, 1998).
Laska, T. et al. 1200 V-trench-IGBT study with square short circuit SOA. In IEEE International Symposium on Power Semiconductor Devices and ICs (ISPSD) 433–436 (IEEE, 1998).
Jaeger, C., Philippou, A., VeIlei, A., Laven, J. G. & Härtl, A. A new sub-micron trench cell concept in ultrathin wafer technology for next generation 1200 V IGBTs. In IEEE International Symposium on Power Semiconductor Devices and ICs (ISPSD) 69–72 (IEEE, 2017). The state-of-the-art Si insulated-gate bipolar transistors with MPT for further reduction of Ron and VCE,sat.
Imperiale, I. et al. Opportunities and challenges of a 1200 V IGBT for 5 V gate voltage operation. In IEEE International Symposium on Power Semiconductor Devices and ICs (ISPSD) 505–508 (IEEE, 2020).
Mori, M. et al. A planar-gate high-conductivity IGBT (HiGT) with hole-barrier layer. IEEE Trans. Electron Dev. 54, 1515–1520 (2007).
Laska, T., Munzer, M., Pfirsch, F., Schaeffer, C. & Schmidt, T. The field stop IGBT (FS IGBT). A new power device concept with a great improvement potential. In IEEE International Symposium on Power Semiconductor Devices and ICs (ISPSD) 355–358 (IEEE, 2000).
Miller, G. & Sack, J. A new concept for a non punch through IGBT with MOSFET like switching characteristics. In IEEE Power Electronics Specialists Conf. (PESC) 21–25 (IEEE, 1989).
Matsudai, T. et al. Advanced 60µm thin 600V punch-through IGBT concept for extremely low forward voltage and low turn-off loss. In IEEE International Symposium on Power Semiconductor Devices and ICs (ISPSD) 441–444 (IEEE, 2001).
Rahimo, M., Kopta, A. & Linder, S. In Novel enhanced-planar IGBT technology rated up to 6.5kV for lower losses and higher SOA capability. In IEEE International Symposium on Power Semiconductor Devices and ICs (ISPSD) 1–4 (IEEE, 2006).
Andenna, M. et al. Soft-punch-through buffer concept for 600–1200 V IGBTs. IET Power Electron. 12, 3874–3881 (2019).
Nakamura, K. et al. Advanced wide cell pitch CSTBTs having light punch-through (LPT) structures. In IEEE International Symposium on Power Semiconductor Devices and ICs (ISPSD) 277–280 (IEEE, 2002).
Takahashi, H., Yamamoto, A., Aono, S. & Minato, T. 1200V reverse conducting IGBT. In IEEE International Symposium on Power Semiconductor Devices and ICs (ISPSD) 133–136 (IEEE, 2004).
Tran, Q. T. et al. RC-GID IGBT – A novel reverse-conducting IGBT with a gate voltage independent diode characteristic and low power losses. In IEEE International Symposium on Power Semiconductor Devices and ICs (ISPSD) 347–350 (IEEE, 2021).
Baliga, B. J. Power semiconductor device figure of merit for high-frequency applications. IEEE Electron. Dev. Lett. 10, 455–457 (1989).
Li, H., Dimitrijev, S., Harrison, H. B. & Sweatman, D. Interfacial characteristics of N2O and NO nitrided SiO2 grown on SiC by rapid thermal processing. Appl. Phys. Lett. 70, 2028–2030 (1997).
Gammon, P. Taking Stock of SiC, Part 1: A Review of SiC Cost Competitiveness and a Roadmap to Lower Cost. PGC Consultancy https://www.pgcconsultancy.com/post/taking-stock-of-sic-part-1-a-review-of-sic-cost-competitiveness-and-a-roadmap-to-lower-costs (2021).
Kimoto, T. & Watanabe, H. Defect engineering in SiC technology for high-voltage power devices. Appl. Phys. Expr. 13, 120101 (2020).
Afanasev, V. V., Bassler, M., Pensl, G. & Schulz, M. Intrinsic SiC/SiO2 interface states. Phys. Status Solidi A 162, 321–337 (1997).
Pensl, G. et al. Alternative techniques to reduce interface traps in n-type 4H-SiC MOS capacitors. Phys. Status Solidi B 245, 1378–1389 (2008).
Chung, G. Y. et al. Effect of nitric oxide annealing on the interface trap densities near the band edges in the 4H polytype of silicon carbide. Appl. Phys. Lett. 76, 1713–1715 (2000).
Tachiki, K., Kaneko, M. & Kimoto, T. Mobility improvement of 4H-SiC (0001) MOSFETs by a three-step process of H2 etching, SiO2 deposition, and interface nitridation. Appl. Phys. Expr. 14, 031001 (2021).
Kimoto, T. et al. Physics and innovative technologies in SiC power devices. In IEEE International Electron Devices Meeting (IEDM) 36.1.1–36.1.4 (IEEE, 2021).
Ni, W. et al. 1700V 34mΩ 4H-SiC MOSFET with retrograde doping in junction field-effect transistor region. In IEEE International Conference on Electron Devices and Solid-State Circuits (EDSSC) 1–3 (IEEE, 2019).
Han, Z. et al. A novel 4H-SiC MOSFET for low switching loss and high-reliability applications. Semicond. Sci. Technol. 35, 085017 (2020).
Matin, M., Saha, A. & Cooper, J. A. A self-aligned process for high-voltage, short-channel vertical DMOSFETs in 4H-SiC. IEEE Trans. Electron. Dev. 51, 1721–1725 (2004).
Nakamura, T. et al. High performance SiC trench devices with ultra-low Ron. In IEEE International Electron Devices Meeting (IEDM) 26.5.1–26.5.3 (IEEE, 2011).
Peters, D. et al. Performance and ruggedness of 1200V SiC-Trench-MOSFET. In IEEE International Symposium on Power Semiconductor Devices and ICs (ISPSD) 239–242 (IEEE, 2017). The state-of-the-art trench gate SiC metal oxide semiconductor field-effect transistors with low Ron,sp and high short-circuit ruggedness.
Takaya, H. et al. A 4H-SiC trench MOSFET with thick bottom oxide for improving characteristics. In IEEE International Symposium on Power Semiconductor Devices and ICs (ISPSD) 43–46 (IEEE, 2013).
Gajewski, D. A. et al. Reliability and standardization for SiC power devices. Mater. Sci. Forum 1092, 179–186 (2023).
Wei, J. et al. Review on the reliability mechanisms of SiC power MOSFETs: a comparison between planar-gate and trench-gate structures. IEEE Trans. Power Electron. 38, 8990–9005 (2023).
Volosov, V. et al. Role of interface/border traps on the threshold voltage instability of SiC power transistors. Solid-State Electron. 207, 108699 (2023).
Lin, W.-C. et al. Investigation of the time dependent gate dielectric stability in SiC MOSFETs with planar and trench gate structures. Microelectron. Reliab. 150, 115141 (2023).
Green, R., Lelis, A. & Habersat, D. Threshold-voltage bias-temperature instability in commercially-available SiC MOSFETs. Jpn. J. Appl. Phys. 55, 04EA03 (2016).
Mimura, T., Hiyamizu, S., Fujii, T. & Nanbu, K. A new field-effect transistor with selectively doped GaAs/n-AlxGa1−xAs heterojunctions. Jpn. J. Appl. Phys. 19, L225 (1980).
Asif Khan, M., Bhattarai, A., Kuznia, J. N. & Olson, D. T. High electron mobility transistor based on a GaN‐AlxGa1−xN heterojunction. Appl. Phys. Lett. 63, 1214–1215 (1993).
Park, S.-H. & Chuang, S.-L. Comparison of zinc-blende and wurtzite GaN semiconductors with spontaneous polarization and piezoelectric field effects. J. Appl. Phys. 87, 353–364 (2000).
Ambacher, O. et al. Two dimensional electron gases induced by spontaneous and piezoelectric polarization in undoped and doped AlGaN/GaN heterostructures. J. Appl. Phys. 87, 334–344 (2000).
Saito, W. et al. Field-plate structure dependence of current collapse phenomena in high-voltage GaN-HEMTs. IEEE Electron. Dev. Lett. 31, 659–661 (2010).
Saito, W. et al. Influence of surface defect charge at AlGaN-GaN-HEMT upon Schottky gate leakage current and breakdown voltage. IEEE Trans. Electron. Dev, 52, 159–164 (2005).
Ando, Y., Makisako, R., Takahashi, H., Wakejima, A. & Suda, J. Dependence of electrical characteristics on epitaxial layer structure of AlGaN/GaN HEMTs fabricated on freestanding GaN substrates. IEEE Trans. Electron. Dev. 69, 88–95 (2022).
Zanoni, E. et al. Reliability and failure physics of GaN HEMT, MIS-HEMT and p-gate HEMTs for power switching applications: Parasitic effects and degradation due to deep level effects and time-dependent breakdown phenomena. In IEEE Wide Bandgap Power Devices and Applications (WiPDA) 75–80 (IEEE, 2015).
Moens, P. et al. On the impact of carbon-doping on the dynamic Ron and off-state leakage current of 650V GaN power devices. In IEEE Intenational Symposium on Power Semiconductor Devices and ICs (ISPSD) 37–40 (IEEE, 2015).
Fu, H., Fu, K., Chowdhury, S., Palacios, T. & Zhao, Y. Vertical GaN power devices: device principles and fabrication technologies — part II. IEEE Trans. Electron. Dev. 68, 3212–3222 (2021).
Jones, E. A., Wang, F. & Ozpineci, B. Application-based review of GaN HFETs. In IEEE Wide Bandgap Power Devices and Applications (WiPDA) 24–29 (IEEE, 2014).
Huang, X., Liu, Z., Li, Q. & Lee, F. C. Evaluation and application of 600 V GaN HEMT in cascode structure. IEEE Trans. Power Electron. 29, 2453–2461 (2014).
Liu, Z., Huang, X., Lee, F. C. & Li, Q. Package parasitic inductance extraction and simulation model development for the high-voltage cascode GaN HEMT. IEEE Trans. Power Electron. 29, 1977–1985 (2014).
Greco, G., Iucolano, F. & Roccaforte, F. Review of technology for normally-off HEMTs with p-GaN gate. Mater. Sci. Semicond. Process. 78, 96–106 (2018).
Xu, N. et al. Gate leakage mechanisms in normally off p-GaN/AlGaN/GaN high electron mobility transistors. Appl. Phys. Lett. 113, 152104 (2018).
Chen, K. J. et al. GaN-on-Si power technology: devices and applications. IEEE Trans. Electron. Devices 64, 779–795 (2017). The state-of-the-art GaN-on-Si power devices in comparison to SiC metal oxide semiconductor field-effect transistors.
Reimers, J., Dorn-Gomba, L., Mak, C. & Emadi, A. Automotive traction inverters: current status and future trends. IEEE Trans. Veh. Technol. 68, 3337–3350 (2019).
Satpathy, S., Das, P. P., Bhattacharya, S. & Veliadis, V. Design considerations of a GaN-based three-level traction inverter for electric vehicles. In IEEE Wide Bandgap Power Devices & Applications (WiPDA) 192–197 (IEEE, 2022).
Holmes, D. G. & Lipo, T. A. Pulse Width Modulation for Power Converters: Principles and Practice Vol. 18 (John Wiley & Sons, 2003).
Aghabali, I. et al. 800-V electric vehicle powertrains: review and analysis of benefits, challenges, and future trends. IEEE Trans. Transp. Electrif. 7, 927–948 (2021). The benefits of increasing battery voltage from 400 V to 800 V.
Zhang, L. et al. Performance evaluation of high-power SiC MOSFET modules in comparison to Si IGBT modules. IEEE Trans. Power Electron. 34, 1181–1196 (2018).
Oswald, N., Anthony, P., McNeill, N. & Stark, B. H. An experimental investigation of the tradeoff between switching losses and EMI generation with hard-switched all-Si, Si-SiC, and all-SiC device combinations. IEEE Trans. Power Electron. 29, 2393–2407 (2014).
Wang, G. et al. Performance comparison of 1200V 100A SiC MOSFET and 1200V 100A silicon IGBT. In IEEE Energy Conversion Congress and Exposition (ECCE) 3230–3234 (IEEE, 2013).
Taha, W. et al. Holistic design and development of a 100 kW SiC-based six-phase traction inverter for an electric vehicle application. In IEEE Transactions on Transportation Electrification https://doi.org/10.1109/TTE.2023.3313511 (2023).
Wang, J., Laird, I., Yuan, X. & Zhou, W. In IEEE Energy Conversion Congress and Exposition (ECCE) 5202–5209 (2021).
Lu, J., Hou, R., Di Maso, P. & Styles, J. A GaN/Si Hybrid T-Type three-level configuration for electric vehicle traction inverter. In IEEE Wide Bandgap Power Devices and Applications (WiPDA) 77–81 (IEEE, 2018).
Millán, J., Godignon, P., Perpiñà, X., Pérez-Tomás, A. & Rebollo, J. A survey of wide bandgap power semiconductor devices. IEEE Trans. Power Electron. 29, 2155–2163 (2014).
Zhao, B., Song, Q., Liu, W. & Sun, Y. Overview of dual-active-bridge isolated bidirectional DC–DC converter for high-frequency-link power-conversion system. IEEE Trans. Power Electron. 29, 4091–4106 (2014). Overview of the dual-active bridge-isolated bidirectional DC–DC converters.
Hurley, W. G., Gath, E. & Breslin, J. G. Optimizing the AC resistance of multilayer transformer windings with arbitrary current waveforms. IEEE Trans. Power Electron. 15, 369–376 (2000).
Mu, M., Li, Q., Gilham, D. J., Lee, F. C. & Ngo, K. D. T. New core loss measurement method for high-frequency magnetic materials. IEEE Trans. Power Electron. 29, 4374–4381 (2014).
Sullivan, C. R. Optimal choice for number of strands in a Litz-wire transformer winding. IEEE Trans. Power Electron. 14, 283–291 (1999).
Kieu, H. P., Lee, D., Choi, S. & Kim, S. GaN-based DC-DC converter with optimized integrated transformer for electrical vehicles. In IEEE Energy Conversion Congress and Exposition (ECCE) 5549–5553 (IEEE, 2021).
Islam, S., Iqbal, A., Marzband, M., Khan, I. & Al-Wahedi, A. M. A. B. State-of-the-art vehicle-to-everything mode of operation of electric vehicles and its future perspectives. Renew. Sustain. Energy Rev. 166, 112574 (2022).
Kempton, W. & Tomić, J. Vehicle-to-grid power implementation: from stabilizing the grid to supporting large-scale renewable energy. J. Power Sources 144, 280–294 (2005). Overview of the vehicle-to-grid bidirectional onboard chargers.
Rahman, S. A. & Persson, E. CoolGaN™ Totem-Pole PFC Design Guide and Power Loss Modeling. Infineon https://www.infineon.com/dgdl/Infineon-Design_guide_Gallium_Nitride-CoolGaN_totem-pole_PFC_power_loss_modeling-ApplicationNotes-v01_00-EN.pdf?fileId=5546d4626d82c047016d95daec4a769a (2019).
Wouters, H. & Martinez, W. Bidirectional onboard chargers for electric vehicles: state-of-the-art and future trends. IEEE Trans. Power Electron. 39, 693–716 (2023).
Etxandi-Santolaya, M., Canals Casals, L. & Corchero, C. Estimation of electric vehicle battery capacity requirements based on synthetic cycles. Transp. Res. D Transp. Environ. 114, 103545 (2023).
GaN-based, 6.6-kW, Bidirectional, Onboard Charger Reference Design. Texas Instruments https://www.ti.com/tool/PMP22650 (2021).
High Power Charging (HPC) Technology. Phoenix Contact https://www.phoenixcontact.com (2024).
Reber, V. E-power: New Possibilities with 800-Volt Charging. Porsche https://www.porscheengineering.com/peg/en/about/magazine/ (2023).
Burress, T. A. et al. Evaluation of the 2010 Toyota Prius Hybrid Synergy Drive System. Oak Ridge National Laboratory https://www.osti.gov/biblio/1007833 (2011).
Kim, D.-H., Kim, M.-J. & Lee, B.-K. An integrated battery charger with high power density and efficiency for electric vehicles. IEEE Trans. Power Electron. 32, 4553–4565 (2017).
Oki, S. & Sato, Y. Nissan LEAF and e-POWER: evolution of motors and inverters. IEEJ J. Ind. Appl. 13, 8–16 (2024).
Chowdhury, S. et al. Enabling technologies for compact integrated electric drives for automotive traction applications. In IEEE Transportation Electrification Conference and Expo (ITEC) 1–8 (IEEE, 2019).
Matallana, A. et al. Power module electronics in HEV/EV applications: new trends in wide-bandgap semiconductor technologies and design aspects. Renew. Sustain. Energy Rev. 113, 109264 (2019).
Li, H. et al. Analysis of SiC MOSFET dI/dt and its temperature dependence. IET Power Electron. 11, 491–500 (2018).
Pahinkar, D. G. et al. Transient liquid phase bonding of AlN to AlSiC for durable power electronic packages. Adv. Eng. Mater. 20, 1800039 (2018).
Luechinger, C. et al. Aluminum–copper ribbon interconnects for power devices. IEEE Trans. Compon. Packag. Manuf. Technol. 7, 1567–1577 (2017).
Lee, H., Smet, V. & Tummala, R. A review of SiC power module packaging technologies: challenges, advances, and emerging issues. IEEE J. Emerg. Sel. Top. Power Electron. 8, 239–255 (2020). Overview of the packaging technologies of SiC power modules.
Weidner, K., Kaspar, M. & Seliger, N. Planar interconnect technology for power module system integration. In IEEE Int. Conf. Integrated Power Electronics Systems (CIPS) 1–5 (2012).
Robles, E. et al. The role of power device technology in the electric vehicle powertrain. Int. J. Energy Res. 46, 22222–22265 (2022).
Chen, C., Luo, F. & Kang, Y. A review of SiC power module packaging: layout, material system and integration. CPSS Trans. Power Electron. Appl. 2, 170–186 (2017).
Gurpinar, E., Chowdhury, S., Ozpineci, B. & Fan, W. Graphite-embedded high-performance insulated metal substrate for wide-bandgap power modules. IEEE Trans. Power Electron. 36, 114–128 (2020).
Ahmed, H. E. et al. Optimization of thermal design of heat sinks: a review. Int. J. Heat Mass Transf. 118, 129–153 (2018).
Behrendt, S., Eisele, R., Scheibel, M. G. & Kaessner, S. Implementation of a new thermal path within the structure of inorganic encapsulated power modules. Microelectron. Reliab. 100–101, 113430 (2019).
Hosoi, T. et al. Performance and reliability improvement in SiC power MOSFETs by implementing AlON high-k gate dielectrics. In IEEE International Electron Devices Meeting (IEDM) 7.4.1–7.4.4 (IEEE, 2012).
Tang, S.-W. et al. High threshold voltage enhancement-mode regrown p-GaN gate HEMTs with a robust forward time-dependent gate breakdown stability. IEEE Electron. Dev. Lett. 43, 1625–1628 (2022).
Kumar, S. et al. 1.2 kV enhancement-mode p-GaN gate HEMTs on 200 mm engineered substrates. IEEE Electron. Dev. Lett. 45, 657–660 (2024).
Wang, Y. & Edmondson, J. Thermal Management for EV Power Electronics 2024–2034: Forecasts, Technologies, Markets, and Trends. IDTechEx https://www.idtechex.com/en/research-report/thermal-management-for-ev-power-electronics-2024-2034-forecasts-technologies-markets-and-trends/1000 (2024).
Al-Hmoud, A., Ismail, A. & Zhao, Y. A high-density 200-kW all Silicon Carbide three-phase inverter for traction applications. In IEEE Applied Power Electronics Conference and Exposition (APEC) 3143–3146 (IEEE, 2023).
NXP. EV Power Inverter Control Reference Design Gen 3 https://www.nxp.com/design/design-center/designs/ev-power-inverter-control-reference-design-gen-3:EV-POWEREVBHD2 (2023).
Stella, F. et al. Design and testing of an automotive compliant 800V 550 kVA SiC traction inverter with full-ceramic DC-link and EMI filter. In IEEE Energy Conversion Congress and Exposition (ECCE) 1–8 (IEEE, 2022).
Chen, Z. et al. An 800V/300 kW, 44 kW/L air-cooled SiC power electronics building block (PEBB). In IEEE Annual Conference of Industrial Electronics Society (IECON) 1–6 (IEEE, 2021).
VisIC Technologies. 800 V 100 kW Motor Inverter Reference Design https://visic-tech.com/100kw-motor-inverter-reference-design-for-800v-power-bus/ (2020).
Adamson, T. et al. An 800-V high-density traction inverter-electro-thermal characterization and low-inductance PCB bussing design. IEEE J. Emerg. Sel. Top. Power Electron. 10, 3013–3023 (2022).
Continental. High Performance Twin Power Module Inverter https://conti-engineering.com/wp-content/uploads/2020/05/High_Performance_Twin_Power_Module_Inverter_EN.pdf (2020).
Zhu, L., Bai, H., Brown, A. & Körner, A. An ultra-high gain current-fed universal auxiliary power module for 400V/800V electric vehicles. In IEEE Applied Power Electronics Conference and Exposition (APEC) 885–891 (IEEE, 2023).
Lee, D.-W., Youn, H.-S. & Kim, J.-K. Development of phase-shift full-bridge converter with integrated winding planar two-transformer for LDC. IEEE Trans. Transp. Electrif. 9, 1215–1226 (2023).
BorgWarner. Explore our Technologies Gen5 High Voltage DC/DC https://cdn.borgwarner.com/docs/default-source/defaultdocument-library/high-voltage-dc-dc-converter-product-sheet.pdf?sfvrsn=3027b23c_12 (2023).
Bel Fuse. 700DNC40-12 Down Converter https://www.belfuse.com/product-detail/power-solutions-custom-value-added-solutions-emobility-700dnc40-down-converter (2023).
Du, X., Diao, F., Zhao, Y., Uvodich, K. & Miljkovic, N. A high-density 5kW 800V to 48VDC/DC converter for vehicle applications. In IEEE Energy Conversion Congress and Exposition (ECCE) 1502–1506 (IEEE, 2021).
Sarnago, H., Lucía, Ó., Menzi, D. & Kolar, J. W. Single-/Three-Phase bidirectional EV on-board charger featuring full power/voltage range and cost-effective implementation. In IEEE Int. Conf. Compatibility, Power Electronics and Power Engineering (CPE-POWERENG) 10227458 (IEEE, 2023).
Pham, P. H., Nabih, A., Wang, S. & Li, Q. 11-kW high-frequency high-density bidirectional OBC with PCB winding magnetic design. In IEEE Applied Power Electronics Conference and Exposition (APEC) 1176–1181 (IEEE, 2022).
Lee, S.-Y., Lee, W.-S., Lee, J.-Y. & Lee, I.-O. High-efficiency 11 kW bi-directional on-board charger for EVs. J. Power Electron. 22, 363–376 (2022).
Kim, H. et al. A single-stage electrolytic capacitor-less EV charger with single- and three-phase compatibility. IEEE Trans. Power Electron. 37, 6780–6791 (2022).
Lee, D.-W., Lee, B.-S., Ahn, J.-H., Kim, J.-Y. & Kim, J.-K. New combined OBC and LDC system for electric vehicles with 800 V battery. IEEE Trans. Ind. Electron. 69, 9938–9951 (2022).
Wei, C., Zhu, D., Xie, H., Liu, Y. & Shao, J. A SiC-based 22kW bi-directional CLLC resonant converter with flexible voltage gain control scheme for EV on-board charger. In PCIM Europe Digital Days; International Exhibition and Conference for Power Electronics, Intelligent Motion, Renewable Energy and Energy Management 1–7 (IEEE, 2020).
Ovartech. 22 kW EV On-board Charger https://www.ovartech.com/wp-content/uploads/2021/08/Ovartech-740V-22KW-OBC-Data-Sheet.pdf (2021).
Innoelectric. On-board Charger https://innolectric.ag/on-board-charger-2-2/?lang=en (2021).